- 1Institute of Marine Science and Technology, Shandong University, Qingdao, China
- 2Joint Lab for Ocean Research and Education at Dalhousie University, Shandong University and Xiamen University, Qingdao, China
- 3Southern Marine Science and Engineering Guangdong Laboratory, Zhuhai, China
- 4State Key Laboratory of Heavy Oil Processing, China University of Petroleum, Beijing, China
- 5Key Laboratory of Tropical Marine Bio-resources and Ecology, South China Sea Institute of Oceanology, Chinese Academy of Sciences, Guangzhou, China
Synechococcus is one group of main primary producers and plays a key role in oceanic carbon fixation and transformation. To explore how the temperature rise affects the bioavailability of Synechococcus-derived dissolved organic matter (SOM) and whether this effect would be altered by the involvement of heterotrophic bacteria, we compared the optical and molecular properties of the SOM of axenic Synechococcus sp. PCC7002 culture (Syn) to that with associated heterotrophic bacteria (SynB) under 15, 18, and 21°C growth temperatures at exponential and decay growth phases. Our results showed that the temperature rise increased the bioavailability of the SOM of both Syn and SynB cultures by lowering the proportion of the hydrogen-poor and double-bond structure-rich humus-like components and highly unsaturated substances, as indicated by the increase of spectral slope ratio (SR) and biological index (BIX) and decrease of humification index (HIX). Moreover, the involvement of heterotrophic bacteria modified the Synechococcus-derived SOM, together with its intracellular dissolved organic matter (DOM) excludes, lowering the SOM bioavailability. Our results indicated that the warming in climate change scenario may enhance the bioavailability of the Synechococcus-derived SOM although it may be tempered by the involvement of heterotrophic bacteria, providing an insight for preservation of the organic carbon pool in global oceans.
Introduction
Synecchococcus is a significant group of photosynthetic carbon sequestration organisms that are widely distributed in coastal and oceanic environments, and is thus of great value in marine ecosystem (Huang et al., 2011; Jiao et al., 2011). Previous studies predicted that ocean warming will promote the importance of Synechococcus in the oceanic carbon cycle through expanding their distribution range and increasing their cell abundance (Morán et al., 2010; Flombaum et al., 2013). The Synechococcus-derived dissolved organic matter (SOM) that is released into surroundings via secretion, natural cell death, viral lysis, and predation (Jiao et al., 2010; Fiore et al., 2015; Xiao et al., 2020) largely contributes to the marine dissolved organic matter (DOM) pool (Jiao et al., 2011; Gontikaki et al., 2013), and is thus one of the main drivers for the oceanic carbon cycle (Dufresne et al., 2008). The ultimate fate of SOM is usually determined by the associated heterotrophic bacteria in the ocean, and the bioavailability of SOM influences its persistence and the development of DOM pools (Jiao et al., 2010; Sarmento et al., 2016). Generally, the bioavailability of DOM refers to the consumed or/and utilized degrees of DOM compounds by associated heterotrophic bacteria, which primarily depends on their chemical composition (Berggren and Giorgio, 2015). Contrary to common belief, the DOM produced by algae did not always have high bioavailability (Koch et al., 2014). For example, a study by Koch et al. (2014) showed that only 20% of organic carbon from marine algae was degraded during the 2-year cultivation. Moreover, the DOM induced by viral lysis from cyanobacteria was detected to have similar optical properties with that from natural deep-ocean, indicating a great possibility that the cyanobacteria-derived DOM directly contributes to the refractory DOM (RDOM) formation (Zhao et al., 2017).
Ocean warming is accelerating, with surface seawater temperature being expected to increase by 1–7°C by the end of this century (Kattsov et al., 2001; Levitus et al., 2005; Gleick et al., 2010; Cheng et al., 2019). Previous studies indicated that global warming enhances the bioavailability of marine DOM (Segschneider and Bendtsen, 2013). The available evidence also indicated the possible effects of ocean warming on the bioavailability of SOM. For instance, many studies reported the temperature rise deeply affected the physiology, biochemical compositions, and carbon fixation of Synechococcus (Fu et al., 2007; Paerl and Huisman, 2008; Flombaum et al., 2013; Harvey et al., 2013; Paul et al., 2018). Furthermore, the elevated temperature has been observed to accelerate the production of phytoplankton fluorescent DOM (FDOM) (Yang et al., 2020). The temperature rise was also observed to be tightly coupled with the amount of extracellular microcystin (Walls et al., 2018), heterocyst glycolipid ketone alcohols (Bauersachs et al., 2014), and fatty acid (Nalley et al., 2018) produced by cyanobacteria. However, it is unclear about the effects of temperature rise on the molecular properties of SOM.
The exudations of both healthy and dead Synechococcus cells contribute to the SOM within surroundings (Agustí et al., 1998; Marañón et al., 2004; López Sandoval et al., 2013; Becker et al., 2014). Approximately, 25–65% of environmental DOM is released by cell lyse, viral lysis, or grazing activity, and is considered as intracellular DOM (Hygum et al., 1997; Myklestad and Swift, 1998; Møller et al., 2003; Malits et al., 2021). Compared with the intracellular DOM released due to cell death, the extracellular DOM secreted during cell growing, e.g., chromophoric DOM (CDOM) and FDOM, were more photolabile, with faster photochemical removal (Bittar et al., 2015). In addition, the molecular weight, double bond equivalents (DBE), hydrogen to carbon ratio (H/C), and modified aromatic index (AImod) of the algae-associated DOM often increase with algae cell growing or decay durations, indicating the transformation from active to refractory DOM (Liu et al., 2021). Apart from that, heterotrophic bacteria also play an important role in both the growth of Synechococcus and transformation of SOM (Zheng et al., 2017, 2019). Experiments assessing the SOM without the effects of heterotrophic bacteria have so far been scarce. In return for utilization of the organic carbon fixed by cyanobacteria, the heterotrophic bacteria benefit them by providing complex and unidentified substances like vitamins and bioavailable trace metals (Amin et al., 2009; Kazamia et al., 2012). Because of this, rendering axenic cyanobacteria is difficult for laboratorial cultures. Therefore, few studies have so far focused on how temperature affects the bioavailability of DOM in Synechococcus, especially under different growth phases and with/without heterotrophic bacteria transformations.
It is of great significance to study the bioavailability change of SOM under elevated temperature when considering its contribution to the oceanic carbon pool. Cyanobacteria like Synechococcus are one group of the dominating photoautotrophic microorganisms in the middle and low latitudinal coastal waters (Flombaum et al., 2013), while the Synechococcus sp. PCC7002 is a model strain and mainly distributed in the coastal waters (Mou et al., 2017, 2018). Therefore, in this study we examined the changes of the properties of DOM from Synechococcus sp. PCC7002 with and without the associated heterotrophic bacteria effects under different growth temperatures (15, 18, and 21°C) at exponential and decay growth phases. This study intended to elucidate how the increased temperature influences the persistence of SOM pool in global oceans.
Materials and Methods
Experimental Design
The marine Synechococcus sp. PCC7002 culture with microbes was obtained from Xiamen University Center for Marine Phytoplankton. In this culture, 30 mainly associated bacteria strains belonging to six species (Supplementary Table 1) were identified with agar 2216 medium. Among them the Halomonas sp. that belongs to class Gammaproteobacteria was dominant and isolated with streak plate methods and used in the following experiments. The axenic Synechococcus sp. PCC7002 was isolated by streaking onto the A+ agar plate and purified by repeatedly culturing in solid and liquid media (Zhang et al., 2021).
To probe how heterotrophic bacteria affect the bioavailability of SOM, we cultured both axenic Synechococcus sp. PCC7002 (Syn) and that with Halomonas sp. (SynB) in A+ medium with the Fe-EDTA, Tris-HCl, and vitamin B12 concentrations being adjusted to 7.5 μM, 4 mM, and 3 nM, respectively (Stevens and Porter, 1980; Ludwig and Bryant, 2011). Such an adjustment was to eliminate the influence of external organic carbon while maintaining the growth of Synechococcus sp. PCC7002. Both the Syn and SynB cultures were grown in 300-ml conical flasks (50-ml cultures) under the temperatures of 15, 18, and 21°C in a light incubator (ZQZYCGF8, Shanghai, China). Continuous growth light was provided by a panel of LEDs (Light Emitting Diodes) on top of the incubator with light intensity of 20 ± 1.0 μmol photons m–2 s–1, that was measured with a microscopically quantum sensor (QRT1, Hansatech, United Kingdom) in a culture flask filled with medium. Such a low growth light was chosen to limit the photodegradation or photo transformation of the Synechococcus-derived SOM, as well as maintain the favorable growth of Synechococcus sp. PCC7002 (Mackey et al., 2017). Under each temperature, 20 replicate flasks for each Syn and SynB culture were maintained for the subsequent determination of growth, SOM absorption, and fluorescence and molecular compositions. In each flask, the initial Synechococcus sp. PCC7002 cell density was adjusted to ∼107 cells ml–1, and extra Halomonas sp. isolated above was co-inoculated in SynB culture to the density of ∼106 cells ml–1 (Zhang et al., 2021). Before inoculating to the Syn culture, the bacteria was pre-cultured 2 days in marine broth 2216 at 33°C and washed twice with the modified A+ medium. All the glassware used in this experiment was pre-combusted at 450°C for 5 h to eliminate the effects of external organic carbon.
Growth Phase Determination
Growth of Synechococcus sp. PCC7002 in Syn and SynB cultures was tracked through monitoring the changes in cell abundance using a flow cytometer (Accuri§ C6, Becton-Dickinson, Franklin Lakes, NJ, United States). Every 1–3 days, 1-ml culture was taken out from random three flasks of Syn and SynB cultures under each temperature, and the cell abundance was measured. In addition, the decay phase can be primarily identified with the visible color changes, i.e., from blue-green to yellow-green.
We identified the exponential growth phase at Days 10–32 in Syn and at Days 14–35 in SynB cultures following Mou et al. (2018), and calculated the specific growth rate (μmax, d–1) with the equation:
where Nt and Nt0 are the culture cell abundance at the time t and t0, respectively.
Dissolved Organic Matter Absorption and Fluorescence Measurements
In the exponential growth phase, random three replicate flasks of Syn and SynB cultures were taken out from the incubator to measure DOM absorption and fluorescence. From each flask, 5 ml culture was taken out and filtrated through a pre-combusted (450°C, 5 h) GF/F filter (Whatman, 25 mm in diameter), and the filtration was collected, dispensed into a brown glass tube, and stored at 4°C after 10-time dilution until later measurements of absorption and fluorescence within 3 days. After sampling, these flasks were discarded to eradicate contamination. At the end of cultivation, such a sampling process was performed again, for the DOM determinations in decay growth phase.
To measure the DOM absorption, the filtration was put into a 1-cm quartz cuvette of spectrophotometer. The DOM absorption spectrum was scanned from 250 to 600 nm with a 1-nm increment, referencing to Milli-Q water. Mean absorptions from 575 to 600 nm were used to correct the effects of scattering and baseline fluctuation (Helms et al., 2008). The absorption coefficient of CDOM was calculated (Bricaud et al., 1981) as:
where aCDOM(λ) is the corrected CDOM absorption coefficient at wavelength λ, ACDOM (λ) is the corrected optical density at wavelength λ, and L is the path length (m).
The spectral slope was calculated using a non-linear least squares fitting routine over the range of 275–295 nm (S275–295) and 350–400 nm (S350–400) (Nvdv, 2002) as:
where λo is a reference wavelength and S is the spectral slope parameter.
The spectral slope ratio (SR) was defined as the ratio of the spectral slope of 275–295 nm to that of 350–400 nm. Generally, the average molecular weight and aromaticity of CDOM are inversely proportional to SR: Lower SR indicates higher aromaticity, while higher SR indicates lower aromaticity and thus easier biological degradation (Helms et al., 2008).
To measure excitation emission matrix (EEM), the DOM sample was dispensed into a 1-cm quartz cuvette and measured using a fluorescence spectrophotometer (Cary Eclipse, Agilent, Santa Clara, CA, United States) with the excitation and emission wavelengths of 250–550 nm and 250–600 nm, respectively. The excitation and emission slit widths were set as 10 nm, with the scan speed of 1,200 nm min–1. Parallel factor analysis (PARAFAC) was performed in MATLAB 2021a with the drEEM toolbox 0.6.3 (Murphy et al., 2013). Moreover, the Milli-Q water was used as blank to calibrate the EEMs data (Lawaetz and Stedmon, 2009; Guo et al., 2011), and all the fluorescent data were presented in Raman Units (RU), from which the humification index (HIX) (Ohno, and Tsutomu., 2002), and biological index (BIX) (Huguet et al., 2009) were calculated.
Molecular Signature Determinations
After measuring DOM absorption and fluorescence, the remaining cultures (∼40 ml in each of three replicate flasks) were mixed together, filtered through a GF/F filter, and collected into a pre-combusted glass bottle. The collected filtrate was extracted through methanol-rinsed and formic acid-acidified PPL solid-period extraction cartridges (500 mg, Agilent Technologies) with the speed of ∼5 ml min–1. After extraction, the cartridges were washed with 15 ml formic acid (Sigma-Aldrich, Darmstadt, Germany, 98%), dried, and stored at -20°C for later DOM molecular signature analysis with a Fourier transform ion cyclotron resonance mass spectrometry (FT-ICR MS). The DOM molecular signature analysis was conducted by a 9.4 Tesla Apex-ultra FT-ICR MS in the Heavy Oil Key Laboratory, School of Chemical Engineering, China University of Petroleum, Beijing, China (He et al., 2020). To determine molecular properties, the DOM in PPL was eluted with 10 ml chromatographically pure methanol (Sigma-Aldrich, Darmstadt, Germany) and injected into instrument at speed of 180 μl h–1. The operating conditions for negative formation were set as: 3.0 kV emitter voltage, 3.5 kV capillary column introduce voltage, and -320 V capillary column end voltage, and the samples were scanned over an m/z range of 200–800. Then, based on the AImod index (Qiao et al., 2020) and H/C, the DOM compounds were grouped into five classes (Seidel et al., 2014): polycyclic aromatics (AImod > 0.66), polyphenols (0.5 < AImod < 0.66), highly unsaturated and phenolic compounds (AImod ≤ 0.5 and H/C < 1.5), aliphatic compounds (1.5 ≤ H/C < 2), and saturated compounds (AImod < 0.5 and H/C > 2). The intensity of molecular formula is also taken into account when calculating the percentage of each class. The DBE values were calculated (Koch and Dittmar, 2006) to characterize the number of double bonds in molecules.
Statistical Analyses
All the parameters were presented with mean and standard deviations (mean ± SD) of three independent biological replicate cultures except for the FT-ICR MS data from a mixture of the three replicates. Paired t-test was performed with OriginPro 2021 software (Origin Lab Corporation, Northampton, MA, United States), and a linear regression was applied to test the correlation of measured parameters to temperature. The significance level was set as p = 0.05.
Results and Discussion
Synechococcus Growth
Growth of Synechococcus sp. PCC7002 in the Syn and SynB cultures under different temperatures is shown in Figure 1. The μmax of Synechococcus in the axenic culture were 0.12 ± 0.02, 0.15 ± 0.04, and 0.17 ± 0.03 d–1 under 15, 18, and 21°C, while that in SynB culture were 0.09 ± 0.02, 0.18 ± 0.02, and 0.18 ± 0.01 d–1, indicating the inhibitory effect of adding bacteria upon the growth merely occurred at low temperature. By contrast, symbiotic heterotrophic bacterial bacteria often promote the growth of algae by providing them with micronutrients like vitamins or bioavailable trace metals (Amin et al., 2009). Such a positive effect is closely related to temperature according to our results. Although adding bacteria showed a limited effect on the growth of Synechococcus at higher temperatures, it indeed induced approximately 50% promotion in cell density at both 18 and 21°C at stationary phase if comparing the cell abundance of Syn culture to that of SynB culture (i.e., 1.21 × 109 ± 2.02 × 107 vs. 2.67 × 109 ± 5.52 × 107 at 18°C; and 1.26 × 109 ± 1.46 × 107 vs. 2.80 × 109 ± 2.13 × 107 at 21°C). Consistent with this finding, a model result by Flombaum et al. (2013) showed that the abundance of Synechococcus increases in the future oceans. It was worth noting that the final cell density of Synechococcus at 15°C was insignificant between Syn and SynB cultures. This may be explained that the heterotrophic bacteria may have activated the growth of Synechococcus through providing them essential micronutrients (Hayashi et al., 2011; Kazamia et al., 2012), but such an activated function just started when temperature is over a threshold of e.g., higher than 18°C (Figure 1).
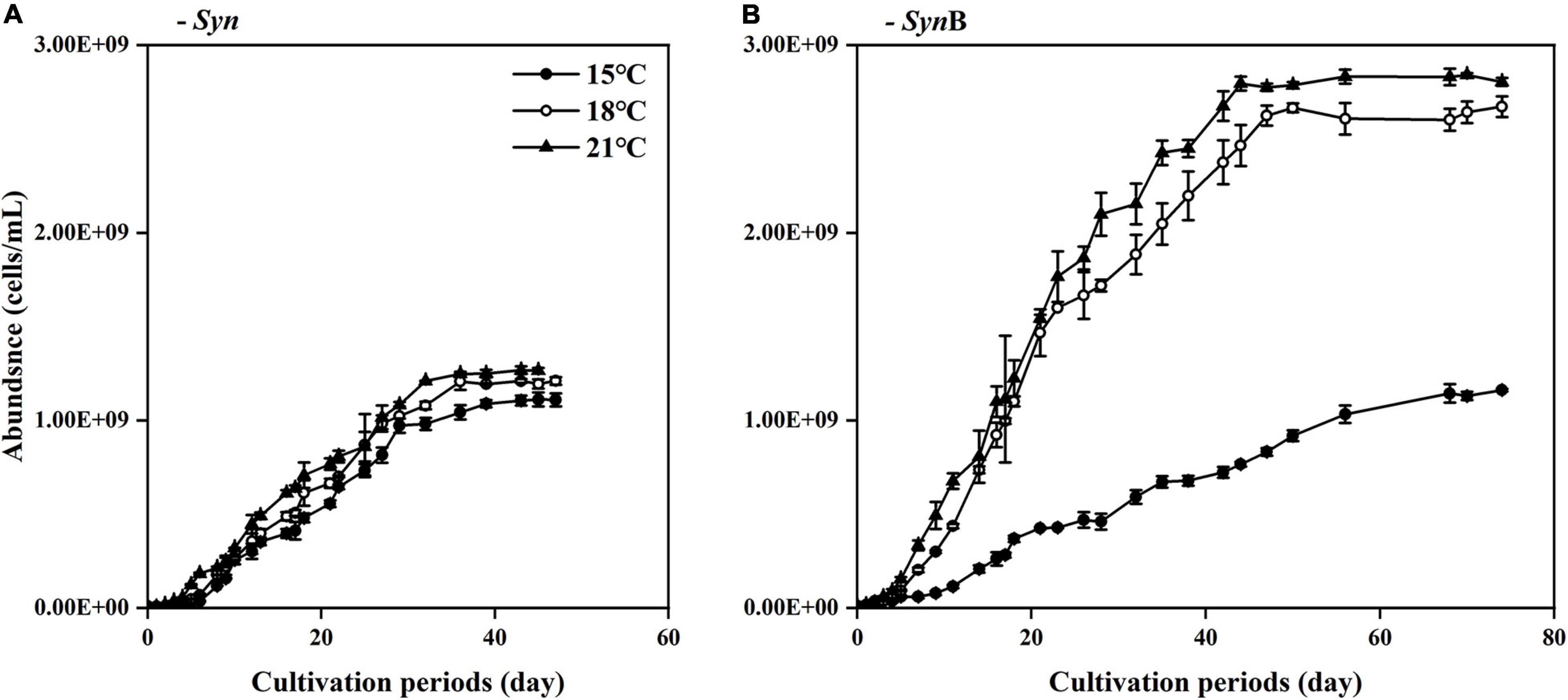
Figure 1. Changes of cell density (indicated by adjusted OD730) of Synechococcus sp. PCC7002 in cultures without [(A), Syn] and with heterotrophic bacteria [(B), SynB] during cultivation periods under temperatures of 15, 18, and 21°C. Points show averages of measurements on three independent cultures and vertical bars show standard deviations (n = 3).
Common Chromophoric Dissolved Organic Matter Properties
Chromophoric dissolved organic matter is an important component of DOM and its vital role in the marine carbon cycle has been widely acknowledged (Yamashita et al., 2010; Andrew et al., 2013). To explore the impacts of temperature on the common CDOM properties of SOM, we measured the absorbance (SR) and fluorescence indicators (HIX and BIX) of the Syn and SynB cultures under three different temperatures (Figure 2), which are well-known to closely correlate with the bioavailability of DOM (Cory and McKnight, 2005; Helms et al., 2008; Huguet et al., 2009; Yuan et al., 2017).
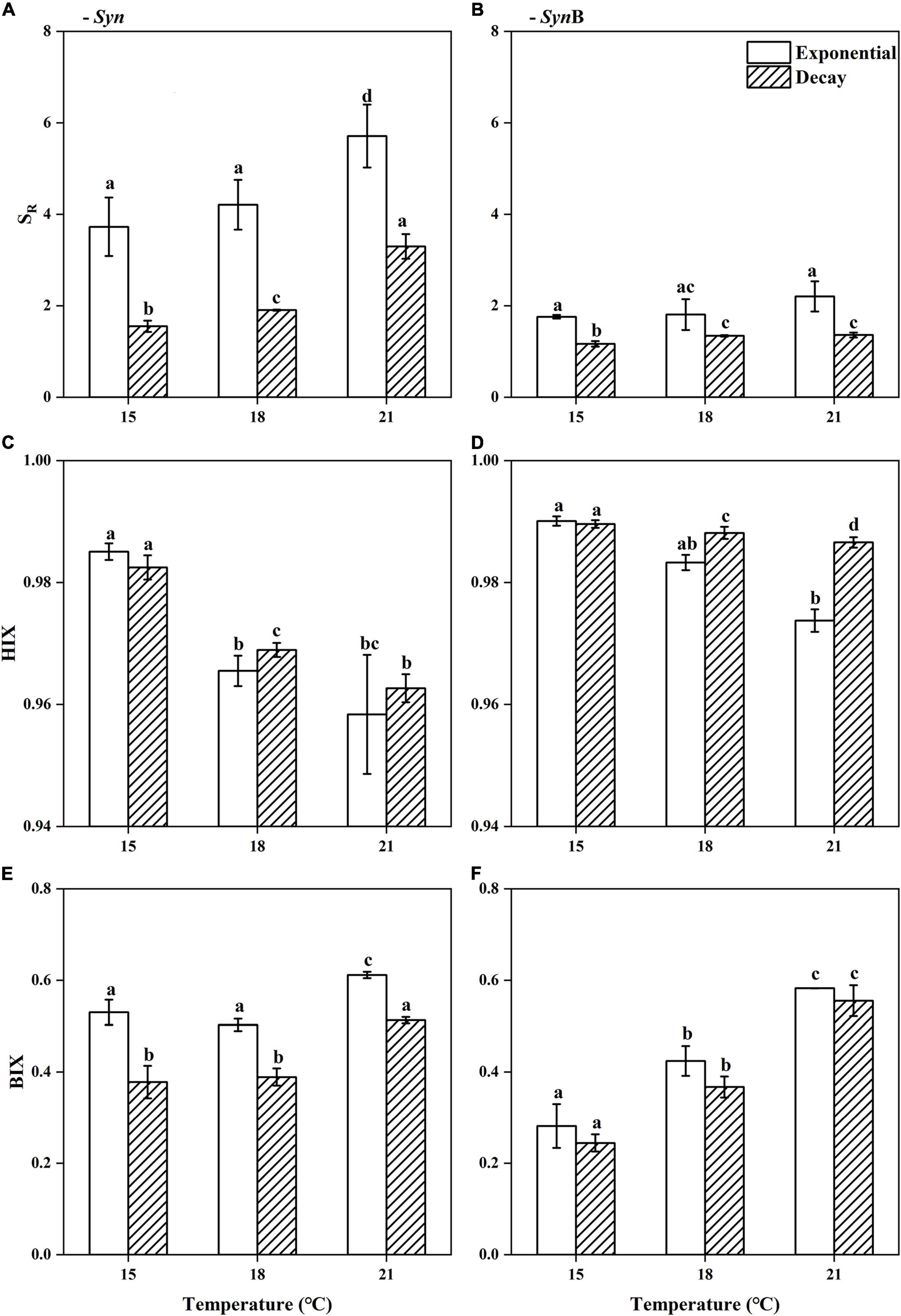
Figure 2. Spectral slope ratio [(A,B), SR], humification index [(C,D), HIX], and biological index [(E,F), BIX] of Synechococcus-derived dissolved organic matter (SOM) at exponential and decay phases, in Synechococcus sp. PCC7002 cultures without [(A,C,E), Syn] and with heterotrophic bacteria [(B,D,F), SynB] under temperatures of 15, 18, and 21°C. Vertical bars indicate standard deviations (n = 3), and different letters on top of bars indicate significant difference (paired t-test, p < 0.05).
In axenic Syn culture, the SR of SOM increased from 3.73 ± 0.64 at 15°C to 5.71 ± 0.69 at 21°C at exponential growth phase, while it increased from 1.55 ± 0.12 to 3.30 ± 0.27 at decay phase (Figure 2A). Higher SR values are generally associated with the lower DOM molecular weight and aromaticity (Helms et al., 2008), while the DOM with lower aromaticity is often less stable and more likely to be utilized by heterotrophic microorganisms (Zhou et al., 2020). Therefore, the elevated temperature may have reduced the molecular weight and aromaticity, leading to the better bioavailability of SOM. Moreover, the SR value at exponential phase was significantly higher than that at decay phase, no matter under what temperatures (Paired t-test, 15°C, t = 6.94, p < 0.05; 18°C, t = 8.82, p < 0.05; 21°C, t = 4.38, p < 0.05), indicating the bioavailability of SOM is higher in former than latter growth phases. A possible explanation is that the more permeability of cell membrane at decay phase allowed the release of intracellular matters like pigments to surroundings, while these metabolites may contribute to the humic-like fluorescent groups (Zhao et al., 2017). It may thus be speculated that the addition of such a humic substance results in the decrease of the bioavailability of SOM. Evidence that elevated temperature enhanced the bioavailability may also be inferred from the HIX and BIX values of SOM, which decreased from 0.99 ± 0.00 to 0.96 ± 0.01 and increased from 0.53 ± 0.03 to 0.61 ± 0.01 with temperature increasing from 15 to 21°C at exponential phase (Figures 2C,E). At decay phase, the HIX was similar to that at exponential phase, but the BIX was significantly lower (paired t-test, 15°C, t = 4.61, p < 0.05; 18°C, t = 6.98, p < 0.05; 21°C, t = 13.48, p < 0.05), and both HIX and BIX presented similar temperature-dependent changes. HIX values correspond to the ratio of fluorescence intensity between humic and protein-like components at long excitation wavelengths (Ohno, and Tsutomu., 2002), and higher HIX values thus indicate the presence of complex molecules like high molecular weight aromatics (Senesi et al., 1991). It is generally believed that the DOM that can persist for a long time in deep sea often contains large amounts of humic substances that are not easily utilized by microorganisms (Zhao et al., 2017); in contrast, protein-like components have a rapid turnover rate and cannot retain for a long time in marine environment (Zhang and Wang, 2017). The BIX characteristics of autochthonous biological activity in water (Huguet et al., 2009) essentially reflects the ratio of fluorescence intensity of humic-like component peaks at short wavelengths to that at long wavelengths. The fluorescence emission spectra usually move toward longer wavelengths with increasing aromaticity (Senesi, 1990). Therefore, the accumulation of more fluorescent substances at short wavelengths means the higher BIX values and the DOM being more easily utilized; the higher BIX values, the more fresh and higher bioavailability of DOM (Cory and McKnight, 2005; Huguet et al., 2009). Given the lower HIX and higher BIX at 21°C condition, the SOM from the cultures under higher temperature have a lower degree of humification and can be retained for a shorter time period. Again, these SOM were inferred from higher bioavailability. The mechanisms underlying this phenomenon need to be studied further. Moreover, consistent with SR, the lower BIX at exponential than decay phases (Figures 2A,E) also indicated the higher bioavailability of SOM in former than latter phases.
All of the above results appeared to suggest that higher temperature favors higher bioavailability of SOM. Does the heterotrophic bacterial biological process change this? As compared to axenic Syn culture, the SR of SOM of non-axenic SynB culture was significantly lower among all temperatures in both exponential and decay growth phases (Paired t-test, t = 5.69, p < 0.05), and with an insignificant increase of increasing temperature (Figure 2B). Similar to SR, the decrease effect by adding heterotrophic bacteria also occurred in the BIX (Figure 2F). The heterotrophic bacteria addition significantly enhanced the BIX at 18 and 21°C, but not at 15°C (Figure 2F), indicating the modification of heterotrophic bacteria on bioactivity of SOM. Consistently, previous studies demonstrated that heterotrophic bacterial processes can make the DOM more resistant to further degradation (Liu et al., 2021). According to Ogawa et al. (2001),Jiao et al. (2010),Jiao et al. (2011), and Benner (2011), most of the SOM modified by heterotrophic bacteria were converted into inorganic carbon and returned to environments, and the others were converted into more complex organic structures and retained in the environments for the extended time-periods, which was also illustrated in this study.
Fluorescent Components of Synechococcus-Derived Dissolved Organic Matter
During calculation process of the above fluorescence parameters, the limited data such as a single point fluorescence signal (e.g., BIX) or the segment of fluorescence signal at a single excitation wavelength (e.g., HIX) were considered, which inevitably introduced into the deviations. The PARAFAC component analysis was thus performed to validate the changes of the bioavailability of SOM. To characterize the structures of SOM, we detailed its fluorescence and identified a total of five main components (C1–C5) from all samples according to EEM-PARAFAC model (Supplementary Figure 1), being detailed in Table 1. Components 1–3 and 5 have been evidenced as humus-like substances (Stedmon et al., 2003; Stedmon and Markager, 2005a,b; Murphy et al., 2008; Yamashita et al., 2008; Zhang et al., 2009), and the Component 4 as protein-like substances (Yu et al., 2015). Components 1–3 are usually considered as terrestrially derived humic substances (Coble, 1996); while some studies have also identified these components as algae-derived DOM (Mou et al., 2017; Zhao et al., 2017), also in this study (Supplementary Figure 1). Component 5 has also been reported to be associated with heterotrophic microbial processes (Zhang et al., 2009). But we found it not only presented in SynB culture, but also in axenic culture of Synechococcus; it suggested the participation of heterotrophic bacteria was not necessary to produce Component 5. Furthermore, component 1 displayed similar optical properties as the fluorescent component BATS1 from deep sea [ex| em: 250 (340)| 460 nm], while the component 5 was similar to component BATS2 [ex| em: 250 (310)| 400 nm] (Zhao et al., 2017). Our results again confirmed the conclusion that Synechococcus is one of an important source of deep-sea autochthonous FDOM (Zhao et al., 2017; Zheng et al., 2020). Previous studies have also identified the protein-like components including tyrosine- and tryptophan-like fluorophores (Stedmon and Markager, 2005a,b), and Component 4 in this study could be identified as autochthonous tyrosine-like fluorophores (Yu et al., 2015). In general, it is speculated that all the fluorescent components identified in this study could be produced by Synechococcus that may do the contribution in field oceans.
Difference in contribution of the five components to total CDOM fluorescence intensity in each DOM sample is shown in Figure 3 (the data quality as indicated by the SD versus mean value of three biological replicates is shown in Supplementary Figure 2). In general, the humus-like fluorescent substances (C1–3 and C5) are dominant in SOM (i.e., 87–95%). A similar result was also reported by Zheng et al. (2020). Furthermore, the humus-like fluorescent components derived from both Syn and SynB cultures were mainly the components 1 and 2 (over 70%). Similar results have also been found in some other Synechococcus cultures, such as Synechococcus CB0101 and Synechococcus sp. YX04-3 (Zhao et al., 2017; Zheng et al., 2019). In Syn culture, the sum of humus-like substances (C1, C2, C3, and C5) significantly decreased with increasing temperature in both exponential (R2 = 0.99, p < 0.05) and decay growth phases (R2 = 0.97, p < 0.05), although no clear trend was observed in each component (Figure 3A). In protein-like component (C4), however, this trend contrarily increased. The protein-like component has simpler cellular structure and could be utilized more easily by heterotrophic bacteria, as compared with the humus-like component (Zheng et al., 2020). Therefore, the increased protein-like component could be responsible for the increase of the bioavailability of SOM at elevated temperature. In addition, reliability of the results about the changes of SOM bioavailability from optical indicators was also considered. However, based on the FDOM data, the reasons why the higher bioavailability of SOM occurred in exponential growth phase and was not modified by heterotrophic bacteria could not be explained at present (Figure 3); this would be further explained by the molecular composition of the SOM identified with FT-ICR-MS.
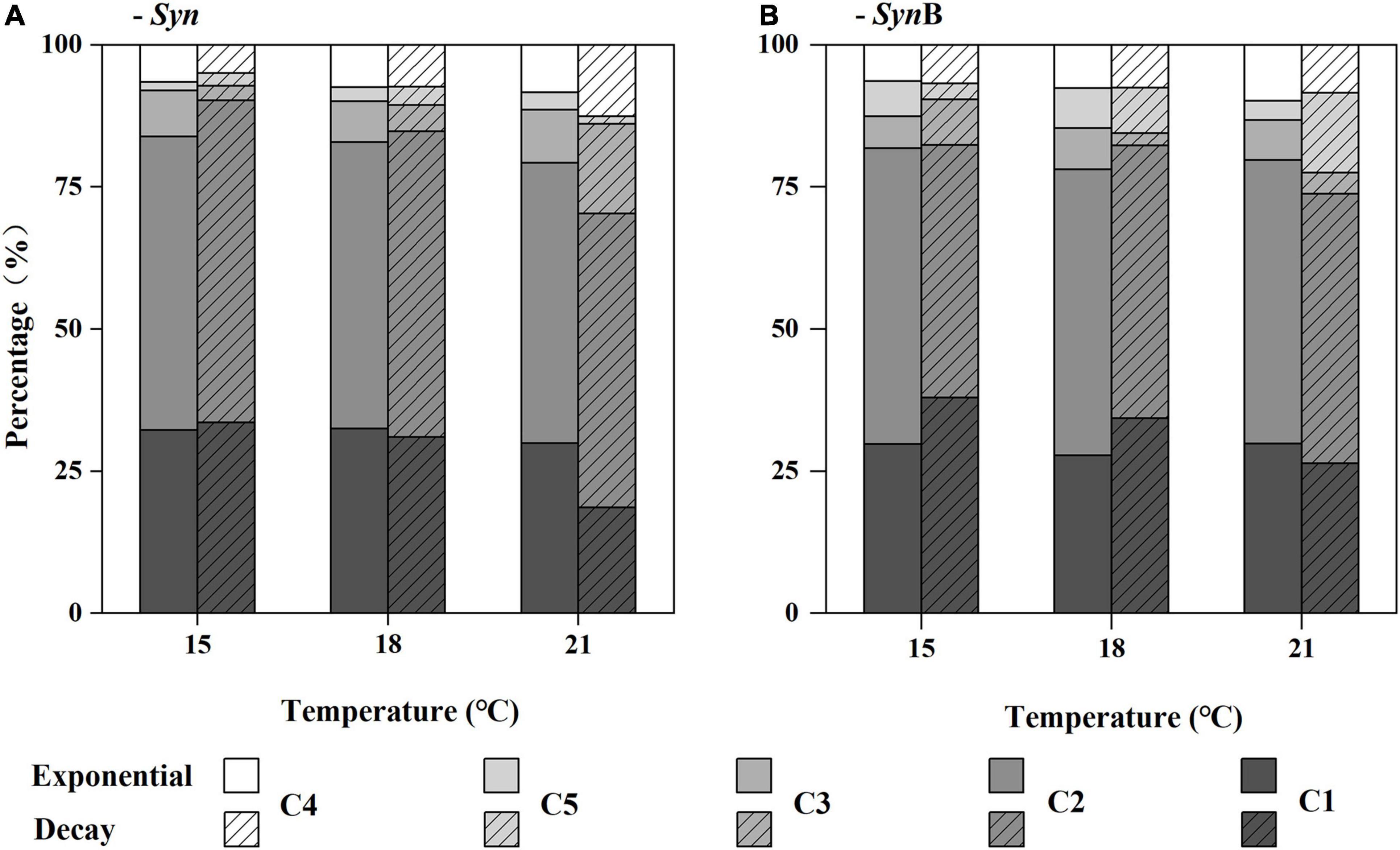
Figure 3. Relative contribution (%) of the main fluorescence components (C1, C2, C3, C4, and C5) to total chromophoric dissolved organic matter (CDOM) fluorescence (Raman Units, RU) of Synechococcus-derived dissolved organic matter (SOM) at exponential and decay phases, in Synechococcus sp. PCC7002 cultures without [(A), Syn] and with heterotrophic bacteria [(B), SynB] under temperatures of 15, 18, and 21°C.
Chemical Composition of Synechococcus-Derived Dissolved Organic Matter
The FT-ICR-MS analysis can provide information about the molecular compositions of DOM (Martínez-Pérez et al., 2017; Zark and Dittmar, 2018) that may indicate its bioavailability through revealing the large diversity of organic compounds (Figure 4 and Supplementary Figure 3). Due to the limitation of extraction efficiency of PPL cartridge, here we just analyzed the extractable and ionizable components of DOM. The 3D Van Krevelen diagram showed the detailed information of SOM molecules in terms of element compositions, i.e., oxygen to carbon ratio (O/C), H/C and nitrogen to carbon ratio (N/C) (Figure 4). Based on the element compositions (CHO, CHNO, CHOS, and CHNOS), the SOM can be classified into four groups. Apart from C, H, and O, they also contained abundant nitrogen and sulfur, consistent with previous studies that reported the DOM released by marine Synechococcus was a substantial source of in situ nitrogen- and sulfur-containing compounds (Zhao et al., 2017; Zheng et al., 2019, 2020). In Syn culture at 15°C temperature, 2,826 and 2,528 molecular formulas were identified in SOM in exponential and decay growth phases, and the numbers were 2,276 and 2,380 at 18°C, and 1,261 and 1,726 at 21°C, respectively (Supplementary Figure 3). The types of identified molecular formulas of SOM were more simplified at higher temperatures, indicating the ocean warming may make the SOM composition more and more simplification. Moreover, the bacteria addition has increased the diversity of SOM molecules (Supplementary Figure 3), suggesting the associated heterotrophic bacteria have contrarily changed this phenomenon.
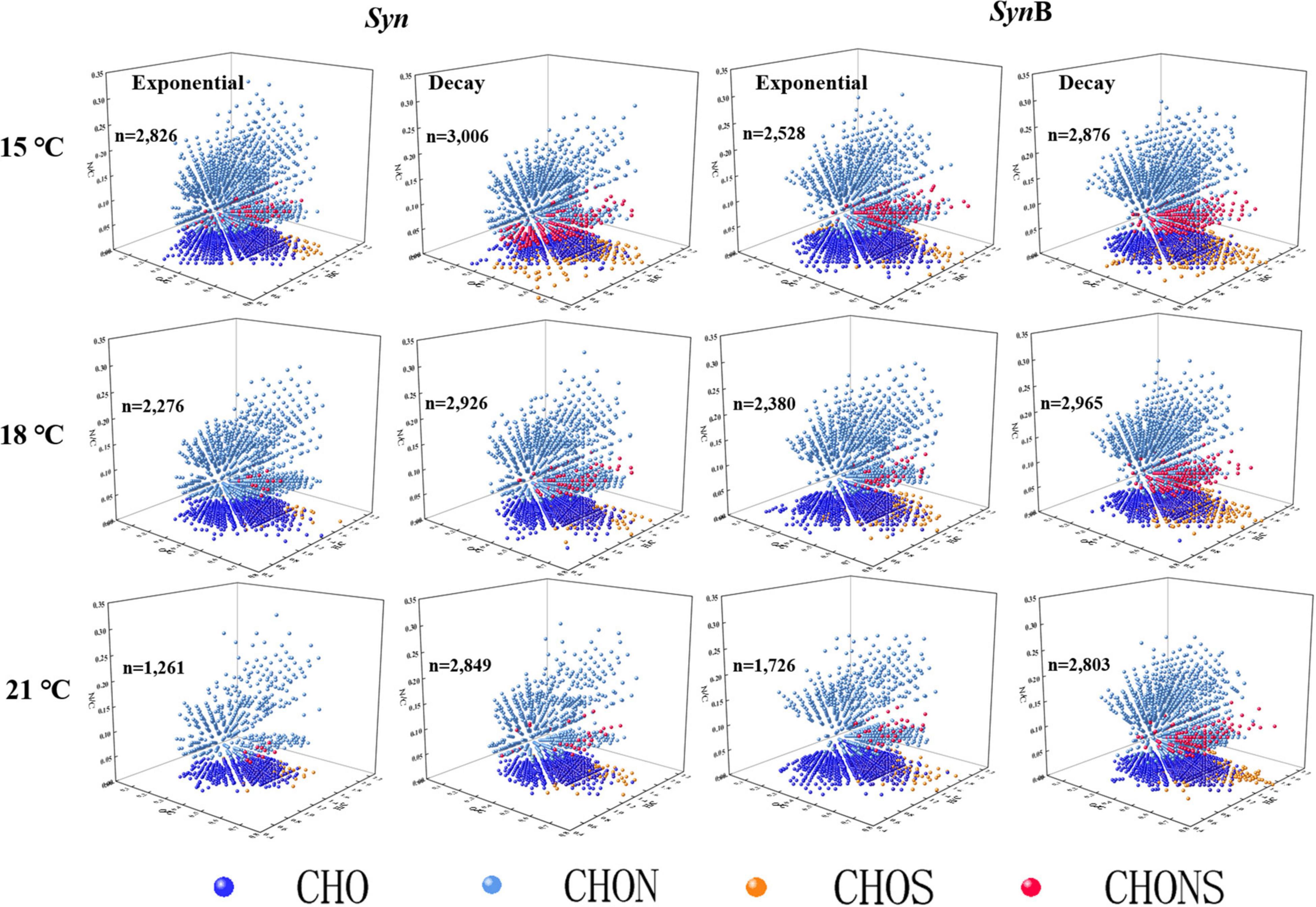
Figure 4. 3D Van Krevelen diagram showing the characteristics of each molecule identified by Fourier transform ion cyclotron resonance mass spectrometry (FT-ICR MS). Each ball represents a molecular formula and “n” is the total number of molecular formulae. Dark blue, light blue, orange, and red colors indicate the formulae containing element CHO, CHON, CHOS, and CHONS, respectively.
To further explore the compositions of SOM, the DOM compounds were divided into five classifications according to AImod value and H/C ratio, among which the sum of highly unsaturated and phenolic compounds and aliphatic compounds accounted for more than 95%, while the remaining (i.e., sum of polycyclic aromatics, polyphenols, and saturated compounds) accounted for less than 5% (Figure 5). According to Liu and co-authors the algal-derived DOM are lack of compounds with the AImod > 0.67 (Liu et al., 2021). Our study further indicated that the proportion of compounds with AImod > 0.5 (polycyclic aromatics and polyphenols) was low in SOM. The saturated compounds were shown with low abundance in various DOM samples (Seidel et al., 2014, 2015; Qiao et al., 2020), consistent with this study. As below, we focused on two more abundant compounds (i.e., highly unsaturated and phenolic compounds and aliphatic compounds). The proportion of aliphatic compounds in Syn cultures increased from 46.14 to 63.24% as temperature increased from 15 to 21°C at exponential growth phase. At decay phase, a similar temperature-dependent increase trend occurred. In contrast, the proportion of highly unsaturated and phenolic compounds decreased with increasing temperature. The modification of cellular fatty acid composition has been widely reported to maintain the normal cell growth across temperatures (Thompson, 1996). Compared with highly unsaturated and phenolic compounds, the aliphatic compounds had higher biodegradability and more rapidly turnover rate (Kellerman et al., 2018). Therefore, it is likely that the increase of aliphatic compounds in SOM caused by elevated temperature was responsible for the increase of its bioavailability. Moreover, the proportion of aliphatic compounds was 1–12% higher in exponential than decay phases among all temperature treatments (Figure 5). It may also explain why the bioavailability of SOM in the exponential growth phase was relatively high due to the lower proportion of highly unsaturated and phenolic compounds and higher aliphatic compounds. This result is consistent with the findings of Liu et al. (2021) that the amount of more recalcitrant compounds increased with the growth and degradation of algal cells. Most of SOM in exponential growth phase was secreted by Synechococcus cells, while in decay growth phase it was a mixture with intracellular substances exuded by cell death. It could be speculated that the bioavailability of intracellular SOM was lower than that being secreted during cell growth. In SynB cultures, the proportion of highly unsaturated and phenolic compounds in culture SynB was about 3–9% higher than that in culture Syn in both growth phases (Figure 5). It is possible that heterotrophic bacterial modifications play an important role in formation of highly unsaturated and phenolic compounds. The heterotrophic bacteria usually prefer to utilize the relatively well-used aliphatic compounds rather than highly unsaturated and phenolic compounds, during which part of aliphatic compounds were transformed into inorganic matters by respiration and the remaining may be modified to other components and retained in cultures (Jiao et al., 2010; Hach et al., 2020). So, the proportion of highly unsaturated and phenolic compounds was higher and that of aliphatic compounds lower in SynB cultures, thus suggesting that heterotrophic bacteria modify the bioavailability of SOM by altering their molecular composition.
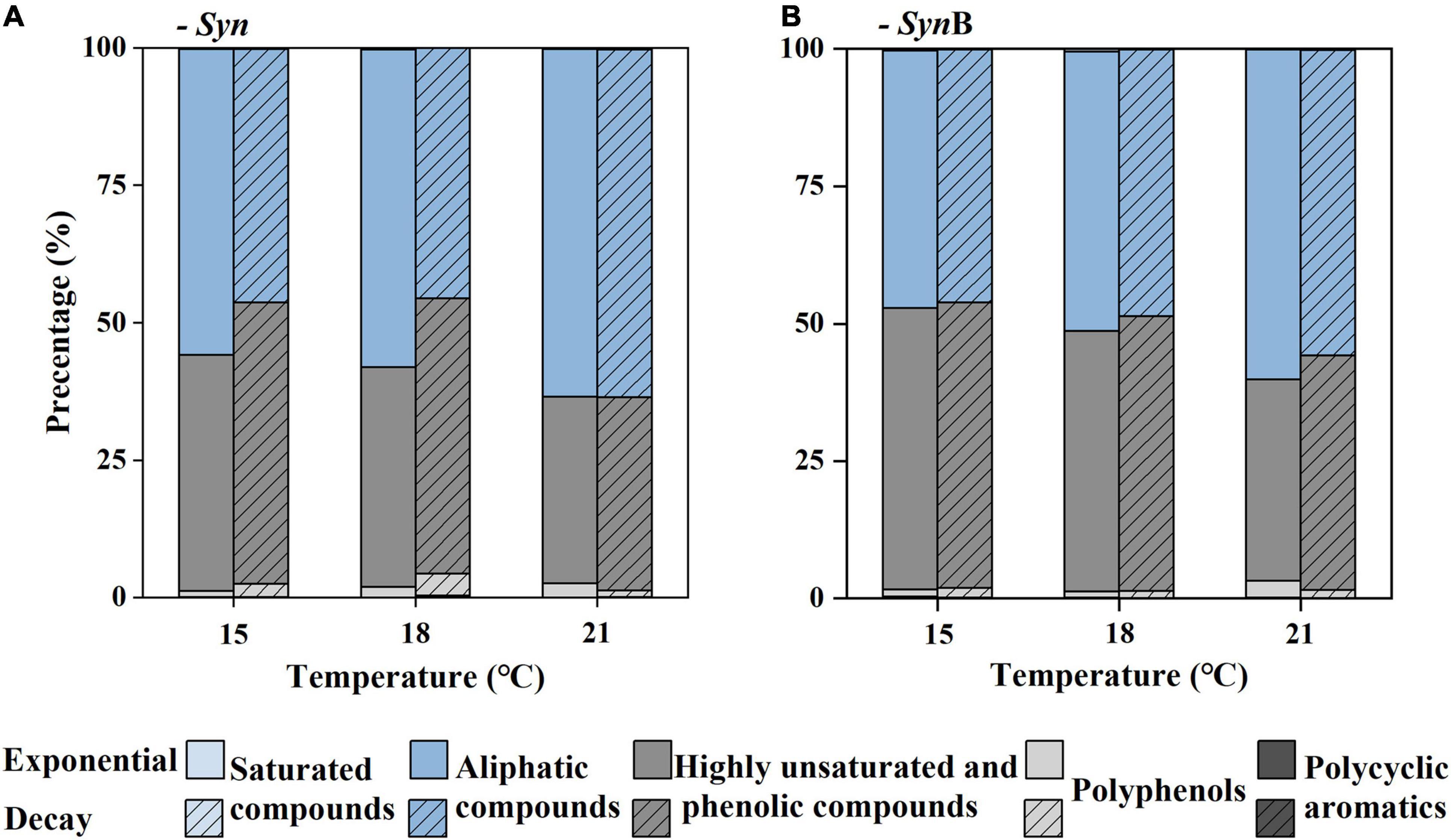
Figure 5. Relative abundance (%) of classification classes based on the modified aromatic index (AImod) values and hydrogen to carbon ratio (H/C) of Synechococcus-derived dissolved organic matter (SOM) at exponential and decay phases, in Synechococcus sp. PCC7002 cultures without [(A), Syn] and with heterotrophic bacteria [(B), SynB] under temperatures of 15, 18, and 21°C. The polycyclic aromatics are defined as AImod > 0.66, polyphenols as 0.5 < AImod < 0.66, highly unsaturated and phenolic compounds as AImod ≤ 0.5 and H/C < 1.5, aliphatic compounds as 1.5 ≤ H/C < 2, and saturated compounds as AImod < 0.5 and H/C > 2.
Overlap and difference of molecular formulas of SOM among different treatments are illustrated in Supplementary Figure 4: the similarities and differences among different growth phases or cultures without or with bacteria (Supplementary Figure 4A) and among different growth temperatures (Supplementary Figure 4B). Approximately two-thirds of molecular formulas of SOM in different cultures or growth phases were shared, and no unique part was observed, while they varied greatly with temperature changes. So, we pooled all the SOM at each temperature together and found the molecular formulas that were shared by three growth temperatures accounted for 54.56% (Supplementary Figure 4B), indicating these molecules were insensitive to temperature changes. The temperature-sensitive molecules accounted for 13.71, 9.78, and 3.59% at 15, 18, and 21°C, respectively, consistent with the result that the most identified molecular formulas presented at 15°C.
By further analyzing the O/C, H/C, AImod, and DBE of these unique molecular formulas, we found these molecular formulas tended to have higher H/C and lower O/C, AImod, and DBE values at higher temperatures (Figure 6). The averaged O/C values for specific molecules were 0.40, 0.39, and 0.36 at 15, 18, and 21°C, while the averaged H/C values were 1.21, 1.34, and 1.48, respectively. Such a decrease in O-containing compounds and increase in H-containing compounds suggested that the bioavailability of organic matters increased and became more well-used by heterotrophic bacteria with increasing temperature (Ohno et al., 2014; Qiao et al., 2020). Moreover, the lower aromaticity in formulas of specific molecules that was higher at higher temperatures (6.71 at 18°C, 6.37 at 21°C) than lower temperatures (8.30 at 15°C) also supported the bioavailability increased with increasing temperatures (Zheng et al., 2019). It was reported that cell membranes typically incorporate more unsaturated compounds at lower temperature, but more saturated substances at higher temperature (Hilditch, 1949; Jacobson et al., 1959). In this study, we confirmed that elevated temperature resulted in the more saturated SOM (Figure 6D). The averaged DBE value decreased from 0.24 to 0.11 from 15 to 21°C. The low value of DBE represents the more saturated structure of molecules and could be considered as an indicative of the substances with high bioavailability (Koch and Dittmar, 2006). In general, SOM at elevated temperature were H-richer, with a higher aromaticity and relatively saturated (lower DBE value), indicating higher bioavailability.
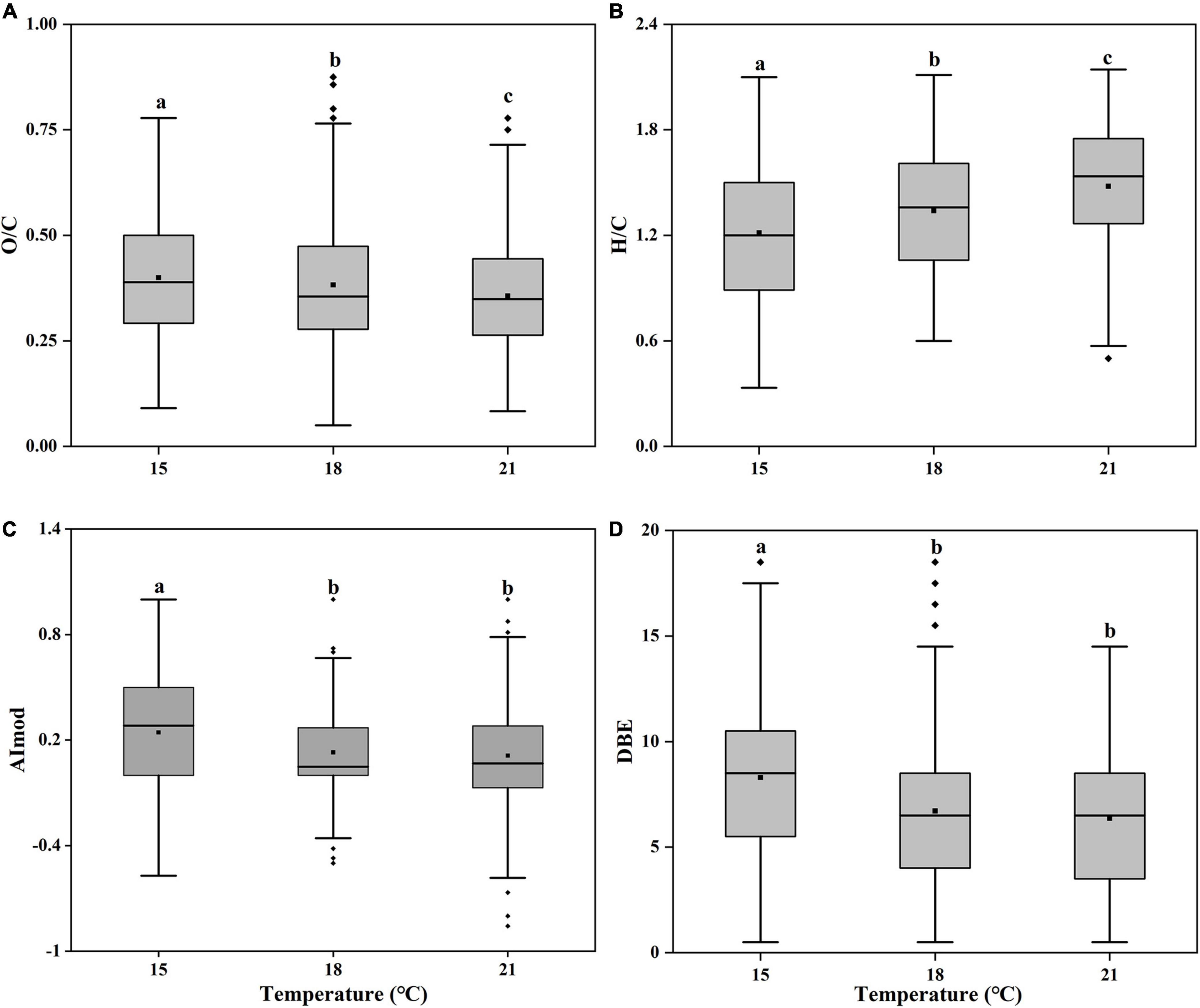
Figure 6. Box plots of pooled oxygen to carbon ratio [(A), O/C], hydrogen to carbon ratio [(B), H/C], modified aromatic index [(C), AImod] and double bond equivalents [(D), DBE] of unique molecular formulas of the Synechococcus-derived dissolved organic matter (SOM) of Synechococcus sp. PCC7002 cultures without and with heterotrophic bacteria at both exponential and decay phases under temperatures of 15, 18, and 21°C. Bottom and top of the box correspond to 25th and 75th percentiles, and the line in box shows the median. Black squares represent the mean values. Vertical lines outside the box represent 10th and 90th percentiles, and filled diamonds denote the outliers. Different letters represent significant differences (paired t-test, p < 0.05).
Conclusion
Heterotrophic bacteria are of great significance to the growth of marine Synechococcus (Zheng et al., 2017); therefore, there have been few studies focused on the SOM that is not modified by bacteria so far. Our study filled this gap and found that the bioavailability of SOM significantly increased with increasing temperatures. Furthermore, through analyzing photochemical properties and molecular compositions, we found the possible reasons for this bioavailability increase of SOM are as follows: (1) the increase of protein-like components; (2) the increase of relatively well-used aliphatic compounds; (3) the increase of hydrogen-containing molecules; and decrease of the unsaturation and aromaticity degrees. In addition, the heterotrophic bacterial processes and intracellular DOM release may decrease the bioavailability, but it did not change the increased trend of the SOM bioavailability with increasing temperatures. Considering the wide distribution of Synechococcus and its abundance increase with increasing temperature (Morán et al., 2010), our results gave a perspective that warming may enhance the bioavailability of certain SOM in global oceans.
Data Availability Statement
The original contributions presented in the study are included in the article/Supplementary Material, further inquiries can be directed to the corresponding author/s.
Author Contributions
JL and GL were in charge of experimental guidance and manuscript revision. JZ was in charge of cultivating experiments and manuscript writing. JZ and XC were in charge of data analysis. DL was in charge of maintenance of laboratory equipment and guidance of cultivating experiments. Molecular determination of the samples was performed by CH and QS. All authors designated in this manuscript contributed to the article and approved the submitted version.
Funding
This work was supported by the National Key Research and Development Program of China (2018YFA0605800), the Key Research and Development Program of Shandong Province (2020ZLYS04), the Innovation Group Project of Southern Marine Science and Engineering Guangdong Laboratory (Zhuhai) (No. 311021006), and National Key Research and Development Program of China (2019YFA0606704).
Conflict of Interest
The authors declare that the research was conducted in the absence of any commercial or financial relationships that could be construed as a potential conflict of interest.
Publisher’s Note
All claims expressed in this article are solely those of the authors and do not necessarily represent those of their affiliated organizations, or those of the publisher, the editors and the reviewers. Any product that may be evaluated in this article, or claim that may be made by its manufacturer, is not guaranteed or endorsed by the publisher.
Acknowledgments
We are very grateful to the two reviewers for their helpful comments and suggestions and would like to thank Yongle Xu for providing the marine Synechococcus sp. PCC7002.
Supplementary Material
The Supplementary Material for this article can be found online at: https://www.frontiersin.org/articles/10.3389/fmicb.2022.838707/full#supplementary-material
References
Agustí, S., Satta, M. P., Mura, M. P., and Benavent, E. (1998). Dissolved esterase activity as a tracer of phytoplankton lysis: Evidence of high phytoplankton lysis rates in the northwestern Mediterranean. Limnol. Oceanogr. 43, 1836–1849. doi: 10.4319/LO.1998.43.8.1836
Amin, S. A., Green, D. H., Hart, M., Küpper, F. C., Sunda, W. G., and Carrano, C. J. (2009). Photolysis of iron–siderophore chelates promotes bacterial–algal mutualism. Proc. Natl. Acad. Sci. U.S.A. 106, 17071–17076. doi: 10.1073/pnas.0905512106
Andrew, A. A., Vecchio, R. D., Subramaniam, A., and Blough, N. V. (2013). Chromophoric dissolved organic matter (CDOM) in the Equatorial Atlantic Ocean: Optical properties and their relation to CDOM structure and source. Mar. Chem. 148, 33–43. doi: 10.1016/J.MARCHEM.2012.11.001
Bauersachs, T., Stal, L. J., Grego, M., and Schwark, L. (2014). Temperature induced changes in the heterocyst glycolipid composition of N2-fixing heterocystous cyanobacteria. Org. Geochem. 69, 98–105. doi: 10.1016/j.orggeochem.2014.02.006
Becker, J. W., Berube, P. M., Follett, C. L., Waterbury, J. B., Chisholm, S. W., Delong, E. F., et al. (2014). Closely related phytoplankton species produce similar suites of dissolved organic matter. Front. Microbiol. 5:111. doi: 10.3389/fmicb.2014.00111
Benner, R. (2011). Biosequestration of carbon by heterotrophic microorganisms. Nat. Rev. Microbiol. 9:75. doi: 10.1038/nrmicro2386-c3
Berggren, M., and Giorgio, P. A. (2015). Distinct patterns of microbial metabolism associated to riverine dissolved organic carbon of different source and quality. J. Geophys. Res. 120, 989–999. doi: 10.1002/2015JG002963
Bittar, T. B., Vieira, A. A. H., Stubbins, A., and Mopper, K. W. (2015). Competition between photochemical and biological degradation of dissolved organic matter from the cyanobacteria Microcystis aeruginosa. Limnol. Oceanogr. 60, 1172–1194. doi: 10.1002/LNO.10090
Bricaud, A., Morel, A., and Prieur, L. (1981). Absorption by dissolved organic matter of the sea (yellow substance) in the UV and visible domains1. Limnol. Oceanogr. 26, 43–53. doi: 10.4319/LO.1981.26.1.0043
Cheng, L., Abraham, J., Hausfather, Z., and Trenberth, K. E. (2019). How fast are the oceans warming? Science 363, 128–129. doi: 10.1126/science.aav7619
Coble, P. (1996). Characterization of marine and terrestrial DOM in seawater using excitation-emission matrix spectroscopy. Mar. Chem. 51, 325–346. doi: 10.1016/0304-4203(95)00062-3
Cory, R., and McKnight, D. (2005). Fluorescence spectroscopy reveals ubiquitous presence of oxidized and reduced quinones in dissolved organic matter. Environ. Sci. Technol. 39, 8142–8149. doi: 10.1021/ES0506962
Dufresne, A., Ostrowski, M., Scanlan, D., Garczarek, L., Mazard, S., Palenik, B., et al. (2008). Unraveling the genomic mosaic of a ubiquitous genus of marine cyanobacteria. Genom. Biol. 9, R90–R90. doi: 10.1186/gb-2008-9-5-r90
Fiore, C. L., Longnecker, K., Soule, M., and Kujawinski, E. B. (2015). Release of ecologically relevant metabolites by the cyanobacterium Synechococcus elongatusCCMP 1631. Environ. Microbiol. 17, 3949–3963. doi: 10.1111/1462-2920.12899
Flombaum, P., Gallegos, J. L., Gordillo, R. A., Rincón, J., and Martiny, A. C. (2013). Present and future global distributions of the marine Cyanobacteria Prochlorococcus and Synechococcus. Proc. Natl. Acad. Sci. U.S.A 110, 9824–9829. doi: 10.1073/pnas.1307701110
Fu, F., Warner, M. E., Zhang, Y., Feng, Y., and Hutchins, D. (2007). Effects of increased temperature and CO2 on photosynthesis, growth, and elemental ratios in marine Synechococcus and Prochlorococcus (cyanobacteria). J. PHYCOL. 43, 485–496. doi: 10.1111/j.1529-8817.2007.00355.x
Gleick, P. H., Adams, R. M., Amasino, R. M., Anders, E., Anderson, D. J., Anderson, W. W., et al. (2010). Climate change and the integrity of science. Science 328, 689–690. doi: 10.1126/science.328.5979.689
Gontikaki, E., Thornton, B., Huvenne, V. A. I., and Witte, U. (2013). Negative Priming Effect on Organic Matter Mineralisation in NE Atlantic Slope Sediments. PLoS One 8:e67722. doi: 10.1371/journal.pone.0067722
Guo, W., Yang, L., Hong, H., Stedmon, C. A., Wang, F., Xu, J., et al. (2011). Assessing the dynamics of chromophoric dissolved organic matter in a subtropical estuary using parallel factor analysis. Mar. Chem. 124, 125–133. doi: 10.1016/J.MARCHEM.2011.01.003
Hach, P. F., Marchant, H., Krupke, A., Riedel, T., Meier, D. V., Lavik, G., et al. (2020). Rapid microbial diversification of dissolved organic matter in oceanic surface waters leads to carbon sequestration. Sci. Rep. 10:13025. doi: 10.1038/s41598-020-69930-y
Harvey, B. P., Gwynn-Jones, D., and Moore, P. (2013). Meta-analysis reveals complex marine biological responses to the interactive effects of ocean acidification and warming. Ecol. Evol. 3, 1016–1030. doi: 10.1002/ece3.516
Hayashi, S., Itoh, K., and Suyama, K. (2011). Growth of the cyanobacterium Synechococcus leopoliensis CCAP1405/1 on agar media in the presence of heterotrophic bacteria. Microb. Environ. 26, 120–127. doi: 10.1264/JSME2.ME10193
He, C., Zhang, Y., Li, Y., Zhuo, X., Li, Y., Zhang, C., et al. (2020). In-House Standard Method for Molecular Characterization of Dissolved Organic Matter by FT-ICR Mass Spectrometry. ACS Omega 5, 11730–11736. doi: 10.1021/acsomega.0c01055
Helms, J. R., Stubbins, A., Ritchie, J., Minor, E., Kieber, D. J., and Mopper, K. (2008). Absorption spectral slopes and slope ratios as indicators of molecular weight, source, and photobleaching of chromophoric dissolved organic matter. Limnol. Oceanogr. 53, 955–969. doi: 10.4319/LO.2008.53.3.0955
Hilditch, T. P. (1949). The Chemical Constitution of Natural Fats. Br. J. Nutr. 3, 347–354. doi: 10.1079/BJN19490048
Huang, S., Wilhelm, S. W., Harvey, H. R., Taylor, K., Jiao, N., and Chen, F. (2011). Novel lineages of Prochlorococcus and Synechococcus in the global oceans. ISME J. 6:285. doi: 10.1038/ismej.2011.106
Huguet, A., Vacher, L., Relexans, S., Saubusse, S., Froidefond, J.-M., and Parlanti, É (2009). Properties of fluorescent dissolved organic matter in the Gironde Estuary. Org. Geochem 40, 706–719. doi: 10.1016/j.orggeochem.2009.03.002
Hygum, B. H., Petersen, J., and Søndergaard, M. (1997). Dissolved organic carbon released by zooplankton grazing activity - a high-quality substrate pool for bacteria. J. Plankton Res. 19, 97–111. doi: 10.1093/PLANKT/19.1.97
Jacobson, B. S., Jaworski, J. G., and Stumpf, P. K. (1959). Fat Metabolism in Higher Plants. Plant Physiol. 49, 497–501. doi: 10.1146/annurev.pp.10.060159.001213
Jiao, N., Herndl, G., Hansell, D. A., Benner, R., Kattner, G., Wilhelm, S., et al. (2010). Microbial production of recalcitrant dissolved organic matter: long-term carbon storage in the global ocean. Nat. Rev. Microbiol. 8, 593–599.
Jiao, N., Herndl, G. J., Hansell, D. A., Benner, R., Kattner, G., Wilhelm, S. W., et al. (2011). The microbial carbon pump and the oceanic recalcitrant dissolved organic matter pool. Nat. Rev. Microbiol. 9, 555–555. doi: 10.1038/nrmicro2386-c5
Kattsov, Z., Joussaume, S., Covey, C., McAvaney, B., Ogana, W., et al. (2001). Climate Change 2001, the Scientific Basis, Chap. 8: Model Evaluation. Contribution of Working Group I to the Third Assessment Report of the Intergovernmental Panel on Climate Change IPCC. Cambridge: Cambridge University Press
Kazamia, E., Czesnick, H., Nguyen, T. T. V., Croft, M., Sherwood, E., Sasso, S., et al. (2012). Mutualistic interactions between vitamin B12 -dependent algae and heterotrophic bacteria exhibit regulation. Environ. Microbiol. 14, 1466–1476. doi: 10.1111/j.1462-2920.2012.02733.x
Kellerman, A., Guillemette, F., Podgorski, D., Aiken, G., Butler, K., and Spencer, R. (2018). Unifying Concepts Linking Dissolved Organic Matter Composition to Persistence in Aquatic Ecosystems. Environ. Sci. Technol. 52, 2538–2548. doi: 10.1021/acs.est.7b05513
Koch, B., and Dittmar, T. (2006). From mass to structure: an aromaticity index for high resolution mass data of natural organic matter. Rapid Commun. Mass Spectrom. 20, 926–932. doi: 10.1002/RCM.2386
Koch, B., Kattner, G., Witt, M., and Passow, U. (2014). Molecular insights into the microbial formation of marine dissolved organic matter: recalcitrant or labile? Biogeosciences 11, 4173–4190. doi: 10.5194/BG-11-4173-2014
Lawaetz, A. J., and Stedmon, C. A. (2009). Fluorescence Intensity Calibration Using the Raman Scatter Peak of Water. Appl. Spectrosc. 63, 936–940. doi: 10.1366/000370209788964548
Levitus, S., Antonov, J., and Boyer, T. (2005). Warming of the world ocean, 1955–2003. Geophys. Res. Lett. 32:L02604. doi: 10.1029/2004GL021592
Liu, Y., Kan, J., He, C., Shi, Q., Liu, Y.-X., Fan, Z.-C., et al. (2021). Epiphytic Bacteria Are Essential for the Production and Transformation of Algae-Derived Carboxyl-Rich Alicyclic Molecule (CRAM)-like DOM. Microbiol. Spectr. 9:e0153121. doi: 10.1128/Spectrum.01531-21
López Sandoval, D. C., Rodríguez-Ramos, T., Cermeño, P., and Marañón, E. (2013). Exudation of organic carbon by marine phytoplankton: Dependence on taxon and cell size. Mar. Ecol. Prog. Ser. 477, 53–60. doi: 10.3354/MEPS10174
Ludwig, M., and Bryant, D. A. (2011). Transcription Profiling of the Model Cyanobacterium Synechococcus sp. Strain PCC 7002 by Next-Gen (SOLiD) Sequencing of cDNA. Front. Microbiol. 2:41. doi: 10.3389/fmicb.2011.00041
Mackey, K., Post, A. F., McIlvin, M. R., and Saito, M. A. (2017). Physiological and proteomic characterization of light adaptations in marine Synechococcus. Environ. Microbiol. 19, 2348–2365. doi: 10.1111/1462-2920.13744
Malits, A., Boras, J. A., Balagué, V., Calvo, E., Gasol, J. M., Marrasè, C., et al. (2021). Viral-Mediated Microbe Mortality Modulated by Ocean Acidification and Eutrophication: Consequences for the Carbon Fluxes Through the Microbial Food Web. Front. Microbiol. 12:635821. doi: 10.3389/fmicb.2021.635821
Marañón, E., Cermeño, P., Fernández, E., Rodríguez, J., and Zabala, L. (2004). Significance and mechanisms of photosynthetic production of dissolved organic carbon in a coastal eutrophic ecosystem. Limnol. Oceanogr. 49, 1652–1666. doi: 10.4319/LO.2004.49.5.1652
Martínez-Pérez, A. M., Osterholz, H., Nieto-Cid, M., Álvarez, M., Dittmar, T., and Álvarez-Salgado, X. A. (2017). Molecular composition of dissolved organic matter in the Mediterranean Sea. Limnol. Oceanogr. 62, 2699–2712. doi: 10.1002/LNO.10600
Møller, E. F., Thor, P., and Nielsen, T. G. (2003). Production of DOC by Calanus finmarchicus, C-glacialis and C-hyperboreus through sloppy feeding and leakage from fecal pellets. Mar. Ecol. Prog. Ser. 262, 185–191. doi: 10.3354/MEPS262185
Morán, X., Lopez-Urrutia, A., Calvo-Díaz, A., and Li, W. K. W. (2010). Increasing importance of small phytoplankton in a warmer ocean. Glob. Chang. Biol. 16, 1137–1144. doi: 10.1111/J.1365-2486.2009.01960.X
Mou, S., Li, G., Li, H., Li, F., Shao, Z., Li, J., et al. (2018). Differential physiological responses of the coastal cyanobacterium Synechococcus sp. PCC7002 to elevated pCO2 at lag, exponential, and stationary growth phases. Sci. China Earth Sci. 61, 1397–1405. doi: 10.1007/s11430-017-9206-5
Mou, S., Zhang, Y., Li, G., Li, H., Liang, Y., Tang, L., et al. (2017). Effects of elevated CO2 and nitrogen supply on the growth and photosynthetic physiology of a marine cyanobacterium, Synechococcus sp. PCC7002. J. Appl. Psychol. 29, 1755–1763. doi: 10.1007/s10811-017-1089-3
Murphy, K. R., Stedmon, C. A., Graeber, D., and Bro, R. (2013). Fluorescence spectroscopy and multi-way techniques. PARAFAC. Anal. Methods 5, 6557–6566. doi: 10.1039/C3AY41160E
Murphy, K. R., Stedmon, C. A., Waite, T. D., and Ruiz, G. M. (2008). Distinguishing between terrestrial and autochthonous organic matter sources in marine environments using fluorescence spectroscopy. Mar. Chem. 108, 40–58. doi: 10.1016/j.marchem.2007.10.003
Myklestad, S. M., and Swift, E. (1998). A new method for measuring soluble cellular organic content and a membrane property. Tm, of planktonic algae. Eur. J. Phycol. 33, 333–336. doi: 10.1080/09670269810001736823
Nalley, J. O., O’Donnell, D. R., and Litchman, E. (2018). Temperature effects on growth rates and fatty acid content in freshwater algae and cyanobacteria. Algal. Res. 35, 500–507. doi: 10.1016/j.algal.2018.09.018
Ogawa, H., Amagai, Y., Koike, I., Kaiser, K., and Benner, R. (2001). Production of Refractory Dissolved Organic Matter by Bacteria. Science 292, 917–920. doi: 10.1126/science.1057627
Ohno, T., Parr, T. B., Gruselle, M. C. I., Fernandez, I. J., Sleighter, R. L., and Hatcher, P. G. (2014). Molecular Composition and Biodegradability of Soil Organic Matter: A Case Study Comparing Two New England Forest Types. Environ. Sci. Technol. 48, 7229–7236. doi: 10.1021/es405570c
Ohno, and Tsutomu. (2002). Fluorescence inner-filtering correction for determining the humification index of dissolved organic matter. Environ. Sci. Technol. 36, 742–746. doi: 10.1021/ES0155276
Paerl, H., and Huisman, J. (2008). Blooms Like It Hot. Science 320, 57–58. doi: 10.1126/science.1155398
Paul, A., Sommer, U., Paul, C., and Riebesell, U. (2018). Baltic Sea diazotrophic cyanobacterium is negatively affected by acidification and warming. Mar. Ecol. Prog. Ser. 598, 49–60. doi: 10.3354/meps12632
Qiao, W., Guo, H., He, C., Shi, Q., and Zhao, B. (2020). Unraveling roles of dissolved organic matter in high arsenic groundwater based on molecular and optical signatures. J. Hazard. Mater. 406:124702. doi: 10.1016/j.jhazmat.2020.124702
Sarmento, H., Morana, C., and Gasol, J. M. (2016). Bacterioplankton niche partitioning in the use of phytoplankton-derived dissolved organic carbon: quantity is more important than quality. ISME J. 10, 2582–2592. doi: 10.1038/ismej.2016.66
Segschneider, J., and Bendtsen, J. (2013). Temperature dependent remineralization in a warming ocean increases surface pCO2 through changes in marine ecosystem composition. Glob. Biogeochem. Cycl. 27, 1214–1225. doi: 10.1002/2013GB004684
Seidel, M., Beck, M., Riedel, T., Waska, H., Suryaputra, I., Schnetger, B., et al. (2014). Biogeochemistry of dissolved organic matter in an anoxic intertidal creek bank. Geochim. Cosmochim. Acta. 140, 418–434. doi: 10.1016/J.GCA.2014.05.038
Seidel, M., Yager, P. L., Ward, N. F. D., Carpenter, E. J., Gomes, H.d.R, Krusche, A. V., et al. (2015). Molecular-level changes of dissolved organic matter along the Amazon River-to-ocean continuum. Mar. Chem. 177, 218–231. doi: 10.1016/j.marchem.2015.06.019
Senesi, N. (1990). Molecular and quantitative aspects of the chemistry of fulvic acid and its interactions with metal ions and organic chemicals : Part II. The fluorescence spectroscopy approach. Anal. Chim. Acta 232, 77–106. doi: 10.1016/S0003-267081226-X
Senesi, N., Miano, T., Provenzano, M. R., and Brunetti, G. (1991). Characterization, differentiation and classification of humic substances by fluorescence spectroscopy. Soil Sci. 152:271. doi: 10.1097/00010694-199110000-00004
Stedmon, C., Markager, S., and Bro, R. (2003). Tracing dissolved organic matter in aquatic environments using a new approach to fluorescence spectroscopy. Mar. Chem. 82, 239–254. doi: 10.1073/pnas.77.10.6052
Stedmon, C. A., and Markager, S. (2005a). Resolving the variability in dissolved organic matter fluorescence in a temperate estuary and its catchment using PARAFAC analysis. Limnol. Oceanogr. 50, 686–697. doi: 10.4319/LO.2005.50.2.0686
Stedmon, C. A., and Markager, S. (2005b). Tracing the production and degradation of autochthonous fractions of dissolved organic matter by fluorescence analysis. Limnol. Oceanogr. 50, 1415–1426. doi: 10.4319/LO.2005.50.5.1415
Stevens, S. E., and Porter, R. D. (1980). Transformation in Agmenellum quadruplicatum. Proc. Natl. Acad. Sci. U.S.A. 77, 6052–6056. doi: 10.1073/pnas.77.10.6052
Thompson, G. A. (1996). Lipids and membrane function in green algae. Biochim. Biophys. Acta. 1302, 17–45. doi: 10.1016/0005-2760(96)00045-8
Walls, J. T., Wyatt, K. H., Doll, J. C., Rubenstein, E. M., and Rober, A. R. (2018). Hot and toxic: Temperature regulates microcystin release from cyanobacteria. Environ. Sci. Technol. 61, 786–795. doi: 10.1016/j.scitotenv.2017.08.149
Xiao, X., Guo, W., Li, X., Wang, C., and Zhang, R. (2020). Viral lysis alters the optical properties and biological availability of dissolved organic matter derived from picocyanobacteria Prochlorococcus. Appl. Environ. Microbiol. 87:e02271–20. doi: 10.1128/AEM.02271-20
Yamashita, Y., Cory, R. M., Nishioka, J., Kuma, K., Tanoue, E., and Jaffé, R. (2010). Fluorescence characteristics of dissolved organic matter in the deep waters of the Okhotsk Sea and the northwestern North Pacific Ocean. Deep Sea Res. Part II Top. Stud. Oceanogr. 57, 1478–1485. doi: 10.1016/J.DSR2.2010.02.016
Yamashita, Y., Jaffeé, R., Maie, N., and Tanoue, E. (2008). Assessing the dynamics of dissolved organic matter (DOM) in coastal environments by excitation emission matrix fluorescence and parallel factor analysis (EEM-PARAFAC). Limnol. Oceanogr. 53, 1900–1908. doi: 10.4319/LO.2008.53.5.1900
Yang, X., Yuan, J., Yue, F., Li, S. L., Wang, B., Mohinuzzaman, M., et al. (2020). New insights into mechanisms of sunlight- and dark-mediated high-temperature accelerated diurnal production-degradation of fluorescent DOM in lake waters. Environ. Sci. Technol. 760:143377. doi: 10.1016/j.scitotenv.2020.143377
Yu, H., Liang, H., Qu, F., Han, Z.-s, Shao, S., Chang, H., et al. (2015). Impact of dataset diversity on accuracy and sensitivity of parallel factor analysis model of dissolved organic matter fluorescence excitation-emission matrix. Sci. Rep. 5:10207. doi: 10.1038/srep10207
Yuan, Z., He, C., Shi, Q., Xu, C., Li, Z., Wang, C., et al. (2017). Molecular Insights into the Transformation of Dissolved Organic Matter in Landfill Leachate Concentrate during Biodegradation and Coagulation Processes Using ESI FT-ICR MS. Environ. Sci. Technol. 51, 8110–8118. doi: 10.1021/acs.est.7b02194
Zark, M., and Dittmar, T. (2018). Universal molecular structures in natural dissolved organic matter. Nat. Commun. 9:3178. doi: 10.1038/s41467-018-05665-9
Zhang, T., and Wang, X. (2017). Release and microbial degradation of dissolved organic matter (DOM) from the macroalgae Ulva prolifera. Mar. Pollut. Bull. 125, 192–198. doi: 10.1016/j.marpolbul.2017.08.029
Zhang, Y., van Dijk, M. A., Liu, M., Zhu, G., and Qin, B. (2009). The contribution of phytoplankton degradation to chromophoric dissolved organic matter (CDOM) in eutrophic shallow lakes: field and experimental evidence. Water Res. 43, 4685–4697. doi: 10.1016/j.watres.2009.07.024
Zhang, Z., Nair, S., Tang, L., Zhao, H., Hu, Z., Chen, M., et al. (2021). Long-Term Survival of Synechococcus and Heterotrophic Bacteria without External Nutrient Supply after Changes in Their Relationship from Antagonism to Mutualism. mBio 12:e0161421. doi: 10.1128/mBio.01614-21
Zhao, Z., Gonsior, M., Luek, J., Timko, S., Ianiri, H., Hertkorn, N., et al. (2017). Picocyanobacteria and deep-ocean fluorescent dissolved organic matter share similar optical properties. Nat. Commun. 8:15284. doi: 10.1038/ncomms15284
Zheng, Q., Chen, Q., Cai, R., He, C., Guo, W., Wang, Y., et al. (2019). Molecular characteristics of microbially mediated transformations of Synechococcus-derived dissolved organic matter as revealed by incubation experiments. Environ. Microbiol. 21(7), 2533–2543. doi: 10.1111/1462-2920.14646
Zheng, Q., Lin, W., Wang, Y., Li, Y., He, C., Shen, Y., et al. (2020). Highly enriched N containing organic molecules of Synechococcus lysates and their rapid transformation by heterotrophic bacteria. Limnol. Oceanogr. 66, 335–348. doi: 10.1002/LNO.11608
Zheng, Q., Wan, Y., Xie, R., Lan, A. S., Liu, Y., Lu, J., et al. (2017). Dynamics of Heterotrophic Bacterial Assemblages within Synechococcus Cultures. Appl. Environ. Microbiol. 84(3), e01517-17. doi: 10.1128/AEM.01517-17
Keywords: dissolved organic matter (DOM), bioavailability, temperature rise, heterotrophic bacteria, exponential and decay phases, Synechococcus sp. PCC7002
Citation: Zhang J, Liu J, Liu D, Chen X, Shi Q, He C and Li G (2022) Temperature Rise Increases the Bioavailability of Marine Synechococcus-Derived Dissolved Organic Matter. Front. Microbiol. 13:838707. doi: 10.3389/fmicb.2022.838707
Received: 18 December 2021; Accepted: 18 February 2022;
Published: 19 April 2022.
Edited by:
Jun Sun, China University of Geosciences, ChinaReviewed by:
Yonghui Zeng, University of Copenhagen, DenmarkPeter Leslie Croot, National University of Ireland Galway, Ireland
Copyright © 2022 Zhang, Liu, Liu, Chen, Shi, He and Li. This is an open-access article distributed under the terms of the Creative Commons Attribution License (CC BY). The use, distribution or reproduction in other forums is permitted, provided the original author(s) and the copyright owner(s) are credited and that the original publication in this journal is cited, in accordance with accepted academic practice. No use, distribution or reproduction is permitted which does not comply with these terms.
*Correspondence: Jihua Liu, liujihua1982@sdu.edu.cn